Here we take a close look at wind and what makes it blow, with the motivation that the more you know about the forces that drive the wind, the better prepared you will be to understand what is going on and be able to make educated guesses at what might happen next. This is the "theory section" we need to build the rest of course.
Away from the several important influences of land, the wind speed and direction we might anticipate (forecast) depends on where we happen to stand within a particular weather pattern. But knowledge builds upon itself: often times the best indicator of where we are in a pattern is the actual direction of the present wind and the way this direction is changing with time. To understand this, requires an understanding of how wind flows around high and Low pressure systems, which we can get to after a quick review of atmospheric pressure itself.
Wind is simply the horizontal motion of air. A 10-knot wind means the air — or a balloon riding along in this air — moves 10 nautical miles in one hour. Air is a fluid with mass. iust as water is, although air is some 800 times lighter (less dense) than water and much more compressible.
Understanding the fluid nature of air and its interaction with fluid water and water vapor is helpful in describing much of practical marine weather. The fluid-fluid interaction between surface winds and the sea makes waves in the sea; a similar interaction between high-altitude winds and the water vapor of clouds makes waves in the clouds. Prominent cloud waves show the direction of the winds aloft just as well as water waves show the direction of surface winds —the direction of winds aloft is extremely valuable to know, because it tells us the direction storms move. Also, storms and frontal systems in the atmosphere behave much like eddies and waves in water.
Air is held to the earth by gravity; if the earth were not heavy enough to provide this attraction, the air would spin off into space from the outward centrifugal force caused by the earth's rotation. Because air is compressible and the holding power of gravity decreases with increasing elevation, most of the atmosphere is compressed into a relatively thin layer at the earth's surface, and this layer itself grows less dense with increasing altitude above the surface. Roughly 80 percent of the air is below 40,000 feet, with some 50 percent of the air below 18,000 feet. This elevation which marks the halfway point through the atmosphere is an important demarcation line in meteorology, because most of the atmospheric disturbances we experience as weather on the surface are confined to this first 18,000 feet of air. The winds above this elevation are stronger and much more persistent than those of the surface; also, the nature of clouds changes — from water vapor to ice crystals — at roughly this elevation.
Besides growing less dense with increasing elevation, the air also grows colder with elevation as less and less of the "atmospheric blanket" is above it to keep it warm. As a rough average, the air temperature drops about 4 F° per 1,000 feet of elevation as you rise through the atmosphere. Distinct cloud bases or ceilings occur at the elevation at which the air temperature has dropped to the dew point of the air.
Although air weighs very little per unit volume, the total weights involved are still impressive. Atypical room contains about 100 pounds of air [note 1 ]. Over each square mile of the earth's surface there is some 30 million tons of air, and these massive amounts of air are not always uniformly distributed over the surface. An uneven distribution of air is what causes the wind. If air flows across an entire state, for example, from the north at 20-knots, all day long, then there must have been very much more air to the north than there was to the south. The amount of air at any place is determined by measuring the atmospheric pressure at that place. One way to describe atmospheric pressure is to simply state how much the air weighs that stands above a given area; a typical value would be 14.7 pounds per square inch, meaning a column of air, one square inch across, extending from the surface straight up to the top of the atmosphere v'/eighs about 14.7 pounds. This is a perfectly valid unit of pressure, but it is more convenient and conventional for tire pressures than for atmospheric pressures — in these units, atmospheric pressures typically vary between 14.4 and 14.8 pounds per square inch.
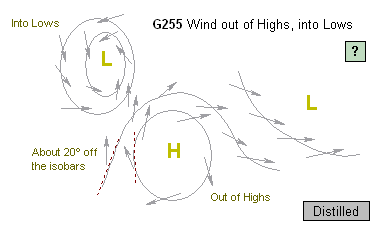
Rather than pounds of air per square inch, atmospheric pressure is usually measured in either inches of mercury (14.7 pounds per square inch = 29.92 inches of mercury) or in millibars (14.7 pounds per square inch = 1013.25 mb). The inches unit derives from the dimensions of a mercury manometer, an instrument used to measure pressure. The height of the mercury column rises or falls according to the atmospheric pressure exerted on the exposed mercury surface. This unit is used in aviation and in commercial radio and TV weather broadcasts.
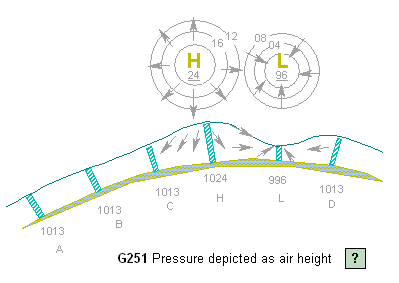
In marine weather broadcasts and all weather maps, on the other hand, the common unit of atmospheric pressure is millibars (mb), and this is the best pressure unit to learn for marine applications. A millibar is a metric unit that derives from a weight of 1 kilogram per square meter of area. To convert inches to millibars use: mb = 33.864 x inches or start with 30.00 inches = 1016 mb and correct this by 1 mb for each 0.03 inches. See atmospheric pressure in the Glossary.
Areas of high and low pressure (called "Highs" and "Lows") are illustrated in G255, which shows schematically the distribution of air over some 1 or 2 thousand miles of ocean. A High is a mound of air; a Low is a depression in the air.
In G251, someone at H measures a higher pressure (1024 mb) than someone at L (996 mb) because there is more air above him.
Since air is a fluid, the natural tendency of air in a pile is to fall down due to gravity, so the first force on air is simply the gravity which forces air away from highs and into Lows —the piles want to fall down; the holes want to fill up. Consequently, air in Highs is always moving out of the High and descending and air in Lows is always moving into the Lows and rising. Near point B in there would be no wind because the air distribution and resulting pressure is equal on all sides of the region. The circles above the High and Low in the picture show how these areas would be represented on a weather map. The circles are contours of equal pressure, called isobars; by convention, they are labeled v'.'ith the last two digits of the pressure they represent and are drawn at 4-mb intervals. Isobars are analogous to elevation contours on topographic maps, and in this schematic picture of the air distribution, they can be thought of as the actual heights of the air mass although actual pressures are also influenced by the density (temperature) of the air as well as its height.
Now, comes a twist to the problem. The driving force of gravity is the same in all horizontal directions, but the actual motion of the air must be described relative to the spherical earth which is rotating below it. The result is called the Coriolis effect: anything that is moving in a straight line above a rotating surface traces out a curoed path on the rotating surface. Think of the path of your footprints on the floor of a merry-go-round as you walk due north from its center as it rotates. You walked straight, but the path as seen by people living on the merry-go-round is curved. (This analogy is, unfortunately, only a poor description of the true Coriolis effect, in which the spherical nature of the earth is critical, but it does show the gist of what takes place. See Coriolis force.)
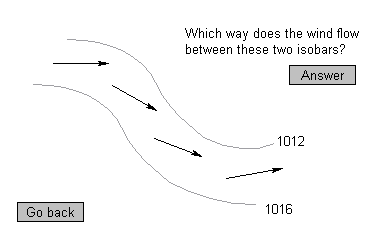
Because of the Coriolis effect, everything (winds, currents, ballistic missiles) in the Northern Hemisphere curves to the right as it progresses and everything moving in the Southern Hemisphere curves to the left. The extent of the curve depends on the speed of motion and on the latitude of the motion. The influence of the Coriolis effect is crucial to most of meteorology and oceanography. Since this effect accounts for a change in motion, it is often called the Coriolis force, even though there is not an actual force involved. Nevertheless, the concept is useful since this "force" can be balanced out by actual forces to account for the net motion of things on the earth.
As air in the Northern Hemisphere is forced out of a High or into a Low by gravity, the Coriolis force bends it to the right (see G255). At higher altitudes in the atmosphere, where no other forces act on the air, the wind continues to curve to the right until the Coriolis force bending it in just balances out the force of gravity which is trying to pull it out. This leaves the air flowinci parallel to the isobars around Lows and Highs (rather than into or out of them) with the wind circulating clockwise around Highs (as it descends) and counterclockwise around Lows (as it rises). Note that it is exactly the same forces that cause the wind to spiral one way around a High and the other way around a Low.
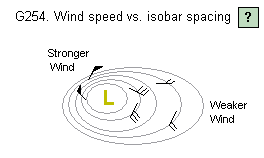
This is the basic rule of how wind flows around Highs and Lows, which is fundamental to predicting winds, but before going on with that, note the rather curious circumstance that this represents. The only true force present is forcing the wind out of the High, yet in fact it is going around the High. It is like a ball set rolling down an inclined plane, which then curves around and starts rolling parallel to the plane without reaching the bottom. This strange affair is the work ot'the Coriolis effect. If we did indeed have a gigantic frictiunless inclined plane attached to the rotating earth, balls would not roll down it, but parallel to the slope. It is a fact to be appreciated without reliance on more common observations.
The schematic description of the air distribution shown in G251 implies the air is more or less uniform in density, but this is only an approximation to real air masses. Due to density variations, a High can have more air in it than neighboring Lows without its being piled higher than the Lows. Cold air is heavier than warm air, so a region of cold air will have a higher pressure than a similar region of warm air of the same height. Nevertheless, the flow from High to Low and the resulting circulation remains the same. The driving force on air can be thought of purely in terms of the pressure on the air, as shown in figure 4. The shaded parcel of air in the High has no net pressure difference on its sides, so there is no motion in that direction, but it has a greater force on the high-pressure side forcing it out than it does on the low-pressure side forcing it in. The net result is the air moves out — and then bends to the right. The same analogy shows how air is forced into Lows.
Although it is gravity that creates the pressure, the picture of pressure forcing the wind is more general. including both the effects of air temperature and elevation. Hence it is better to think of the pressure gradient as the basic driving force of the wind rather than the gravity which creates the pressure.
Because pressure is the eventual driving force on wind,
the effect of gravity on wind is usually called the pressure gradient force. As the name implies, the steeper the gradient (slope) of the pressure, the stronger the force and the faster the wind. Just as a ball will accelerate faster down a steep slope than it will down a gentle slope, winds in a deep Low— or in the steepest part of a Low — will be much faster than those in a weak or shallow Low. Steep pressure gradients show up on a weather map as closely spaced isobars, just as a steep slope does on a topographic map (G254).
So far, we have discussed winds at hiciher elevations. As wind blu'.'vs along the earth's surface, hov'.'ever, there is an additional friction force acting on it that alters both its speed and direction. Again, friction on air is an llusive concept, but recalling its fluid nature, think of •io>"' fast water might run down an inclined piece of sandpaper compared to an inclined piece of glass. Both li-iids are affected by friction. Friction forces always 'esist the direction of motion. As the pressure forces the wind out of a High, frictional drag on the surface slows it jov"n; as the Coriolis force bends the wind to the right, :he surface friction opposes this change and prevents he wind from bending all the way to the right as it does at higher elevations without friction.
rhe result is that surface wind speeds are slower than :hose of higher winds, and the direction of surface wind Joes not exactly follow the isobars around the High but 'emains pointed slightly out of the High, as shown in Igure 6. The same thing happens with Lows; as the wind enters a Low, surface drag prevents it from bending completely to the right, so it ends up pointed slightly into the Low, rather than precisely around the Low. As a rule of thumb, the angle that surface winds point out of a High or into a Low is some 15° to 30° off the isobars, depending on the sea state and cuivature of the isobars. On land where the friction is larger, this angle is more like 30° to 45°
Although it might seem a minor point, this difference in wind speed and direction as a function of elevation — or more generally, as a result of friction — is quite important in practical weather watching and forecasting. To best describe these points, we need to review a few terms. Winds are named according to the direction from which they come. A north wind, blows from the north, toward the south; a southwest wind is coming from the southwest; etc. Likewise, a sea breeze blows from the sea, toward the land; a land breeze blows from the land, toward the sea. Wind shifts are also given names.
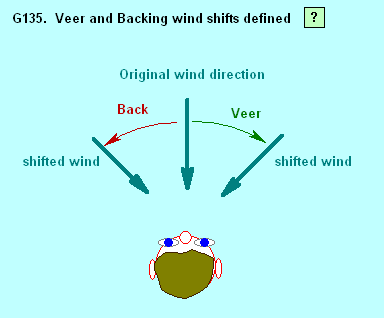
A wind shift to the right is called a veer; a wind shift to the left is called a backing shift, as shown in G135. A north v"ind that shifts to a northeast wind has veered; if it shifted to a northwest wind, it has backed. A southwest v'.'ind veers when shifting to the west and backs when shifting to the south. These definitions apply to all wind directions and to any location, both Northern and Southern Hemispheres. The term "clocking" is the same as veering, but it is not as tidy a term. Directions relative to the wind are also named. Windward means looking into the wind; leeward is looking downwind. A lee shore is one you see to leeward, that the wind blows onto.
Referring to G168, the wind due east of a High would be a north wind of, say, 30 knots at the elevation of cumulus clouds, but a north-northwest wind of 20 knots on the surface, due to the frictional drag. In other words,winds aloft are veered relative to surface winds, and this is true of all winds around Highs or Lows. One consequence of this is the motion of cumulus clouds.
Because they are riding long in the Higher winds, they do not move in same direction as the surface wind. If you face the surface wind, you should see cumulus clouds approaching from slightly to the right. For the same reasons, squalls or thunderstorms you see directly to windvord are not actually headed your way. It is typically the guys some 30° or so to the right of windward that will cross your path.
Wind gusts are another example of this effect. Gusts are caused by a vertical instability in the air. They are caused by a vertical instability in the air. They are usually just large patches of higher air that have temporarily swooped down to the surface. Since the gust wind is coming from above where there is no friction, the gust wind is stronger in speed and veered in direction relative to the average wind you experience between gusts. In short, when the wind gusts, it will typically shift to the right.
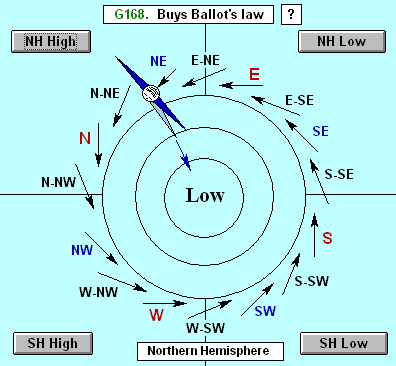
Another consequence of friction is its contribution to the typical change in the wind near a relatively flat shoreline. Because water has less friction than land, winds in general are stronger over water than they are over land. It is not uncommon to have twice the wind speed over a large body of water as you have at the same time over nearby land — an important practical point to keep in mind as you pull away from a sheltered or inland anchorage. When these frictional forces change at the shoreline, the wind direction also changes, and this wind shift usually starts qradually some distance offshore (see G267). Witl'i more friction on land, the wind is backed over the land relative to over the water.
This effect is obviously more important to sailors than to powerboaters or paddlers, but it can be stated as: any wind that flows diagonally toward or avi/ay from a shoreline when well offshore will tend to flow more perpendicular to the shoreline as you get closer to land. See ART-4 on wind and terrain.
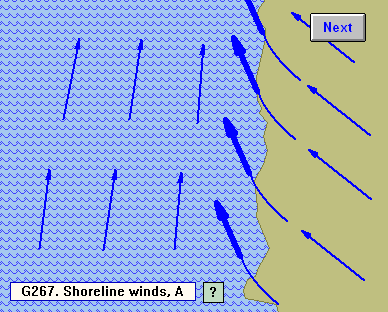
Beside the fact that winds circle opposite ways around Highs and Lows, there is a much more important difference between these two pressure systems: low pressure always brings bad weather and high pressure usually brings fair weather. More to the point, Lows have strong winds; Highs have weak winds. To see how this comes about, requires a closer look at the forces involved — and a bit of arm waving.
As wind circles a High it has two driving forces acting on it in opposite directions; the pressure gradient force pushing it out of the High and the Coriolis force bending it into the High. If the air travels in a circle around the High, these tv'.'o forces must be "balanced," but they cannot be exactly equal in strength. If they were exactly equal, the wind would have no net force on it and it would move in a straight line, not a circle. "Balanced forces" has a special meaning vi'hen things move in a circle. To move in a circle, the inward, or center-seeking, force must be sufficiently larger than the outward force to keep bending it around in a circle. In any circular motion, the net center-seeking force is called the centripetal force; it is always equal to the inward force minus the outward force. For wind circling a High:
Centripetal force = Coriolis force - Pressure gradient force.
This equation can be transposed to get an expression for the pressure gradient present when winds circle a High:
Pressure gradient (High) = Coriolis force - Centripetal force.
When wind circles a Low, however, the inward force is the pressure gradient (pushing the wind into the Low) and the outward force is the Coriolis force. Or, for wind circling a Low:
Centripetal force = Pressure gradient force – Coriolis force, and Pressure gradient (Low) = Coriolis force + Centripetal force.
The important difference between these gradient equations for Highs and Lows is the sign (+ or -) of the centripetal force. In Lows it adds to the gradient; in Highs it subtracts. This difference is important because the curvature forces do not vary at the same rate. The strength of the Coriolis force increases in direct proportion to the wind speed, but the strength of the centripetal force required for circular motion increases in proportion to the square of the wind speed. Winds of low velocity can indeed circle Highs, but as the wind speed increases, the required centripetal force increases faster than the Coriolis force does, so it cant bend it into a circle. Since steep circular Highs cannot exist, there cannot be strong winds circling a High. The natural tendency of a circular High is to flatten out.
Lows, on the other hand, are just the opposite. Both terms in the gradient are positive, so the faster the wind goes, the steeper the gradient becomes. In the case of Lows, whenever the wind starts moving in a circle, it accelerates. Lows are destined to have strong winds.
Once set in motion, they must run their course of continuing to deepen until they run out of fuel. Since their fuel is warm moist air, this happens whenever they run onto land where the air is drier or when they stop moving and consume all the warm moist air that is available.
As a rule, the faster a Low moves, the more intense its winds are. In the summer, Lows travel at some 10 to 20 knots on the average; in the winter they travel typically 20 to 30 knots. In any season, Lows can stop (called stationary Lows), and in the winter they can reach speeds as high as 60 knots. Throughout the mid-latitudes (30° to 60°), Lows move eastward across the oceans. In the tropics, low pressure disturbances tend to move westward — a difference that can be traced back to the Coriolis effect.
All bad weather systems are low pressure systems. A storm is a large circular Low; hurricanes and other tropical disturbances are small, intense Lows; frontal systems are long narrow Lows; and squalls are very localized localized Lows beneath tall cumulonimbus clouds. Besides strong winds, Lows bring clouds and rain because the air is always rising in a Low. Clouds form when the rising air cools to the dew point. When the air becomes saturated, or the weight of the water and ice in the clouds can no longer be supported by the up draft of air, it starts to rain. In Highs, on the other hand, the air is descending, so any clouds that happen into a High will evaporate as they heat on the way down. Highs are characterized by clear skies and light winds.
Properties of Highs and Lows are compared in 6153.
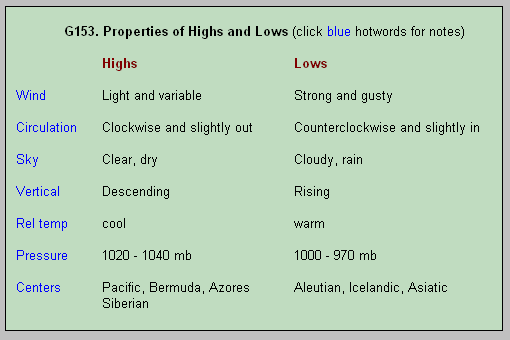
We conclude this discussion with two West Coast examples of wind behavior that are fully understandable on the basis of the way winds flow around Highs and Lows. Later issues will cover other examples that illustrate the importance of these principles. Figure 9 shows a typical weather pattern in the Pacific Northwest: a high pressure ridge along the coast with an offshore Low headed in. The dashed line is the path of the system that will pass eastward over the coastal mariner during a period of about one day. The expected winds can be figured by tracing this path to the west. The ridge provided clear skies and light winds from the northwest. As the Low approaches, the wind will back around to the west, southwest, and on to the south at the leading edge of the Low. The isobars are closer near the Low, so the winds will be strong by the time they arrive from the south. As the storm passes, the wind veers back to the west.
Along this coast and in many other parts of the world, the first sign that bad weather is soon to replace fair wind from the northwest, will be the onset of a backing shift in the wind. For this reason, it is valuable to note the precise wind direction when underway — say, from 285° in the morning, shifting to 265° by noon — rather than being aware only that the wind is generally westerly.
Farther south along this coast (G158) the summer coastal winds are often enhanced by the interaction between a High and a Low. The figure shows a thermal trough of low pressure that builds up over the heated land (when land heats, the air above it grows less dense and rises forming a Low). The isobars between this trough and the prevailing Pacific High then pack up to form a steep gradient that brings strong north to northwest v'.'inds along the coast. Since both systems are stable, this pattern can last for several days. The long fetch involved produces large seas in this pattern. It is not uncommon to have steady 30-knot winds with 14-foot seas for several days when this pattern develops. Because it is on the edge of a High, this wind can come with clear skies, although it is just as likely to be in thick fog in this area.
From "Weather Trainer" by David Burch
Visit blog to discuss this artilce |